How rockets work: A complete guide
- Get link
- X
- Other Apps
By Giles Sparrow, All About Space magazine
Rockets of all kinds are still our only way of reaching space — but how exactly do they work?
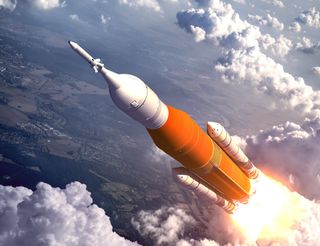
Rockets are our species' best way of escaping the atmosphere of Earth and reaching space. But the process behind getting these machines to work is far from simple. Here's what you need to know about getting a rocket into space.
How rockets lift off
Writers and inventors have dreamt of exploring the universe beyond Earth for centuries, but the real challenges of traveling into space only became clear in the 19th century. Experimental balloon flights showed that Earth's atmosphere thins out rapidly at high altitudes, and so even before powered flight became a reality, engineers knew that devices that create a forward or upward force by pushing against a surrounding medium like air — such as wings and propellers — would be of no use in space.
Another problem was that combustion engines — machines such as steam or gasoline engines that generate power by burning fuel in the oxygen from Earth's atmosphere — would also fail in airless space.
Fortunately, a device that solved the problem of generating force without a surrounding medium had already been invented — the rocket. Initially used as weapons of war or in fireworks, rockets generate a force in one direction, called thrust, by the principle of action and reaction: exhaust fumes released by explosive chemicals are pushed out of the back of the rocket at high speed, and as a result the rocket is pushed in the other direction, regardless of any surrounding medium, NASA explains in this primer (pdf).
The key to using rockets in space is to carry a chemical called an oxidant that can perform the same role as oxygen in Earth's air and enable the fuel to combust.
The first person to seriously study the rocket's potential for space travel, Russian schoolteacher and amateur scientist Konstantin Tsiolkovsky, first published his conclusions in 1903. He correctly identified the launch as one of the biggest challenges — the moment where the rocket has to carry all the fuel and oxidant it needs to reach space — as its weight is at a maximum and a huge amount of thrust is needed just to get it moving.
As the rocket gets underway it sheds mass through its exhaust, so its weight is reduced and the same amount of thrust will have a greater effect in terms of accelerating the rest of the rocket. Tsiolkovsky came up with various rocket designs and concluded that the most efficient setup was a vertically launched vehicle with several 'stages' — each a self-contained rocket that could carry the stages above it for a certain distance before exhausting its fuel, detaching and falling away. This principle, still widely used today, reduces the amount of dead weight that needs to be carried all the way into space.
Tsiolkovsky devised a complex equation that revealed the necessary thrust force needed for any given rocket maneuver, and the "specific impulse" — how much thrust is generated per unit of fuel — needed for a rocket to reach space. He realized that the explosive rocket propellants of his time were far too inefficient to power a space rocket, and argued that liquid fuels and oxidants, such as liquid hydrogen and liquid oxygen, would ultimately be needed to reach orbit and beyond. Although he did not live to see his work recognized, Tsiolkovsky's principles still underpin modern rocketry.
Taking flight
Rockets must delicately balance and control powerful forces in order to make it through Earth's atmosphere into space.
A rocket generates thrust using a controlled explosion as the fuel and oxidant undergo a violent chemical reaction. Expanding gases from the explosion are pushed out of the back of the rocket through a nozzle. The nozzle is a specially shaped exhaust that channels the hot, high-pressure gas created by combustion into a stream that escapes from the back of the nozzle at hypersonic speeds, more than five times the speed of sound.
Isaac Newton's third law of motion states that every action has an equal and opposite reaction, so the "action" force that drives the exhaust out of the rocket nozzle must be balanced by an equal and opposite force pushing the rocket forward. Specifically, this force acts on the upper wall of the combustion chamber, but because the rocket motor is integral to each rocket stage, we can think of it acting on the rocket as a whole.

Although the forces acting in both directions are equal, their visible effects are different because of another of Newton's laws, which explains how objects with greater mass need more force to accelerate them by a given amount. So while the action force rapidly accelerates a small mass of exhaust gas to hypersonic speeds each second, the equal reaction force produces a far smaller acceleration in the opposite direction on the far greater mass of the rocket.
As the rocket gains speed, keeping the direction of motion closely aligned with the direction of thrust is critical. Gradual adjustments are needed to steer the rocket towards an orbital trajectory, but a severe misalignment can send the rocket whirling out of control. Most rockets, including the Falcon and Titan series and the Saturn V moon rocket, steer using gimballed engines, mounted so that the entire rocket motor can pivot and vary the direction of its thrust from moment to moment. Other steering options include using external vanes to deflect the exhaust gases as they escape the rocket engine — most effective with solid-fueled rockets that lack a complex motor — and auxiliary engines, such as small thruster rockets mounted on the sides of the rocket stage.
How a rocket's motors work
Modern rocket motors have come a long way from fireworks, the first in rocket history. Relatively simple solid rockets, most often used as boosters to provide extra thrust at launch, still rely on the same basic principle of igniting a tube containing a combustible mix of fuel and oxidant. Once ignited, a solid rocket will continue to burn until its fuel is exhausted, but the rate at which fuel is burnt — and therefore the amount of thrust — can be controlled by changing the amount of surface exposed to ignition during different times in the rocket's flight.
This can be done by packing the fuel/oxidant mix with a hollow gap down the center, running along the length of the rocket. Depending on the profile of this gap, which may be circular or star-shape, for instance, the amount of exposed surface will change during the flight.
This article is brought to you by All About Space.
All About Space magazine takes you on an awe-inspiring journey through our solar system and beyond, from the amazing technology and spacecraft that enables humanity to venture into orbit, to the complexities of space science.
The more widespread liquid-fueled rockets are far more complex. Typically, they involve a pair of propellant tanks — one each for the fuel and the oxidant — connected to a combustion chamber through a complex maze of pipes. High-speed turbopumps driven by their own independent motor systems are used to deliver liquid propellant into the chamber through an injection system. The rate of supply can be throttled up or down depending on requirement, and fuel can be injected as a simple jet or a fine spray.
Inside the combustion chamber an ignition mechanism is used to begin combustion — this may be a jet of high-temperature gas, an electric spark or a pyrotechnic explosion. Rapid ignition is critical — if too much fuel/oxidant mixture is allowed to build up in the combustion chamber than a delayed ignition can generate enough pressure to blow the rocket apart, a catastrophic event that rocket engineers laconically refer to as a "hard start" or "rapid unscheduled disassembly" (RUD).
The detailed design of a liquid rocket stage can vary a lot depending on its fuel and other requirements. Some of the most efficient propellants are liquefied gases such as liquid hydrogen, which is only stable at very low temperatures — around minus 423 degrees Fahrenheit (minus 253 degrees Celsius). Once loaded aboard the rocket, these cryogenic propellants must be stored in heavily insulated tanks. Some rockets avoid the need for an ignition mechanism using hypergolic propellants that ignite spontaneously on contact with each other.
Interplanetary travel
Rockets are the key to exploring our solar system, but how do they go from orbit to deep space?
The first stage of any spaceflight involves launch from Earth's surface into a relatively low orbit around 124 miles (200 km) up, above the vast majority of the atmosphere. Here gravity is almost as strong as it is on the surface, but friction from Earth's upper atmosphere is very low, so if the uppermost stage of the rocket is moving fast enough it can maintain a stable, circular or elliptical trajectory where the pull of gravity and the vehicle's natural tendency to fly off in a straight line cancel each other out.
Many spacecraft and satellites travel no further than this low Earth orbit (LEO), but those destined to leave Earth entirely and explore the wider solar system need a further boost in speed to reach escape velocity — the speed at which they can never be pulled back by our planet's gravity.
The escape velocity at Earth's surface — 6.9 miles per second (11.2 km/s) — is about 50% faster than the typical speeds of objects in LEO. It gets lower at a greater distance from Earth, and probes bound for interplanetary space are often first injected into elongated or elliptical orbits by a carefully timed burst of thrust from an upper-stage rocket, which may remain attached to the spacecraft for the rest of its interplanetary flight. In such an orbit the spacecrafts' distance from Earth can range from hundreds to thousands of miles, and its velocity will also vary, reaching a maximum when the spacecraft is closest to Earth — a point called perigee — and slowing down further out.

Surprisingly, however, the critical rocket burn used to escape into interplanetary space is usually made when the spacecraft is near perigee. This is due to the so-called Oberth effect, an unexpected property of rocket equations that means a rocket is more efficient when it is moving at higher velocity.
One way to understand this is that burning a spacecraft's fuel allows the engine to utilize not only its chemical energy, but also its kinetic energy, which is greater at higher speeds. On balance, the additional rocket thrust needed to reach escape velocity from a low altitude at higher speed is less than that needed to escape from a high altitude when moving at a lower speed.
Spaceflight engineers and mission planners often refer to the "Delta-v" required to accomplish a specific flight maneuver, such as a change in orbit. Strictly speaking, the term Delta-v means change in velocity, but engineers use it specifically as a measure of the amount of impulse, or thrust force over time, needed to accomplish a maneuver. Broadly speaking, missions are planned around a "Delta-v budget" — how much thrust they can generate for how long using the spacecraft's onboard fuel supplies.
Sending a spacecraft from one planet to another with minimum Delta-v requirements involves injecting it into an elliptical orbit around the sun, called a Hohmann transfer orbit. The spacecraft travels along a segment of the elliptical path that resembles a spiral track between the orbits of the two planets, and requires no further thrust along its journey. On arrival at its target object it may use gravity alone to enter its final orbit, or it may require a burst of rocket thrust in the opposite direction — usually accomplished by simply turning the spacecraft around in space and firing the motor — before it can achieve a stable orbit.
Join our Space Forums to keep talking space on the latest missions, night sky and more! And if you have a news tip, correction or comment, let us know at: community@space.com.
Comments
Post a Comment